Exploring the Composition of DNA Ladder Rungs
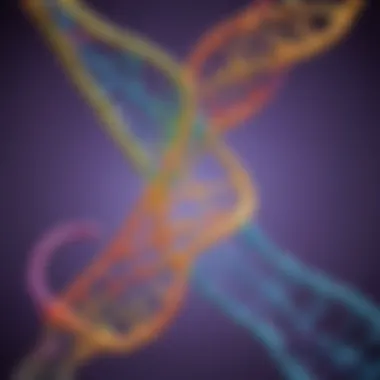
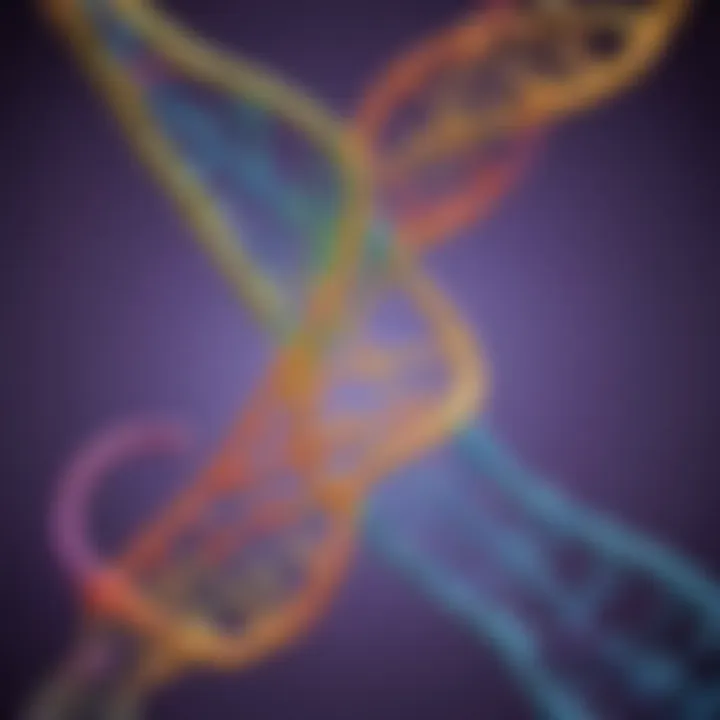
Intro
Understanding the intricate structure of DNA is akin to unraveling a complex tapestry of life. At its core, DNA consists of two long strands that twist around each other, forming what is often called the double helix. But what truly brings this structure to life are the ladder rungs made of nitrogenous bases. These small building blocks hold the secrets of heredity, mutations, and even biotechnological advancements.
This article aims to dive into the detailed composition of these ladder rungs, discussing the various nitrogenous bases that play a role in forming the genetic code. The relationships between these bases and their biological significance will be explored, shedding light on how they contribute to the greater structure of DNA. Overall, the journey through the realms of deoxyribonucleic acid is not just a study of molecules but an exploration of the blueprint for all living creatures.
Prelude to DNA Structure
Understanding the structure of DNA is akin to cracking a complex code that holds the key to life itself. As the foundational element of genetics, DNA determines a myriad of characteristics in living organisms, from the color of a person's eyes to the intricate workings of metabolic processes. In this article, we will examine the structural composition of DNA, specifically focusing on its vital ladder rungs formed by nitrogenous bases.
The significance of this topic cannot be overstated. Grasping the basic principles of DNA structure can reveal the underlying mechanics of heredity, evolution, and even biotechnology. For educators, parents, and curious minds alike, exploring how these components interact encourages a richer understanding of biological sciences.
Basic Overview of DNA
At its core, DNA, or deoxyribonucleic acid, is a molecule composed of two long strands that twist around each other, forming a double helix. Each strand is built from a backbone of sugar and phosphate groups, while the rungs of the ladder, so to speak, are made from pairs of nitrogenous bases. This arrangement creates a stable yet flexible structure, crucial for maintaining the integrity of genetic information.
DNA appears in every living cell, orchestrating who we are at our most fundamental level. The simplest analogy might be to think of DNA as a recipe book, with each recipe corresponding to a trait or function within an organism. It's fascinating how just four types of bases — adenine, thymine, cytosine, and guanine — are responsible for coding the complex array of life.
Role of DNA in Living Organisms
The role of DNA extends far beyond the notion of heredity. It serves as the blueprint for building proteins, which are essential for almost every biological function. Here are some key points regarding the central roles DNA plays in life:
- Genetic Blueprint: DNA carries the instructions for organism development, growth, reproduction, and functioning.
- Protein Synthesis: The sequence of bases in DNA guides the production of RNA, which in turn translates to the creation of proteins, critical for cellular functions.
- Heredity: DNA is passed from parents to offspring, ensuring that traits are inherited across generations.
- Evolution: Through mutations and genetic variation, DNA enables evolution by providing material for natural selection to act upon.
In a nutshell, DNA is more than just a repository of genetic information; it's the foundational system that organizes life as we know it.
"Understanding DNA is not just for scientists; it is essential for anyone curious about the building blocks of life."
This deep dive into the structural aspects of DNA will guide us through its ladder rungs, enhancing our appreciation for this marvelous molecule.
Understanding the Rungs of the DNA Ladder
Understanding the rungs of the DNA ladder is crucial in grasping the entire fabric of genetic material. These rungs, made up of nitrogenous bases, form the very core of what makes up DNA. Without a clear comprehension of how these bases operate and interact, one might find it hard to fully appreciate the biological narratives DNA encodes. Furthermore, these bases go beyond mere inclusion; they are central to life's processes, offering structural integrity and functional fidelity.
The stability and specificity of the DNA double helix are all down to how these rungs lock together, adhering to pairing rules. This interaction stands out as a splendid example of nature's elegance, where simplicity manifests in a complex biological dance. Understanding these concepts not only paves the way for appreciating genetics but also illuminates the pathways to comprehending mutations, heredity, and even advancements in biotechnology. It sets the stage for deeper discussions about genetic codes and maybe even life itself.
What Comprises the Rungs of DNA?
The rungs of the DNA ladder are composed primarily of nitrogenous bases. These bases can be thought of as the "letters" in the genetic alphabet, responsible for encoding the instructions that guide cellular function. There are four main bases in DNA: adenine, thymine, cytosine, and guanine. The way these bases pair up follows specific rules, creating a structure that not only gives DNA its shape but also its stability.
Each base has unique properties that influence how they bond with one another. Adenine pairs with thymine, while cytosine bonds with guanine. This predictable pairing is what provides consistency in the genetic message. Moreover, the arrangement of these bases is what ultimately determines the instructions for building proteins, influencing traits and functions in living organisms.
Types of Nitrogenous Bases
Adenine
Adenine plays a significant role as one of the building blocks of DNA. As a purine base, it is characterized by a double-ring structure that allows it to pair effectively with thymine through hydrogen bonds. This pairing is not just a convenient match; it’s a critical aspect of DNA stability. Adenine's prominence in DNA is largely due to its ability to form strong hydrogen bonds, which are vital for maintaining the integrity of the double helix. However, it can sometimes appear in alternative forms in RNA, like adenosine, which adds a layer of complexity to its functions, especially in cellular processes.
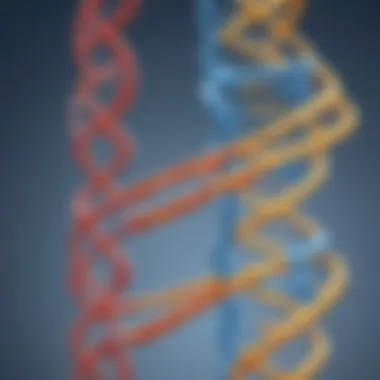
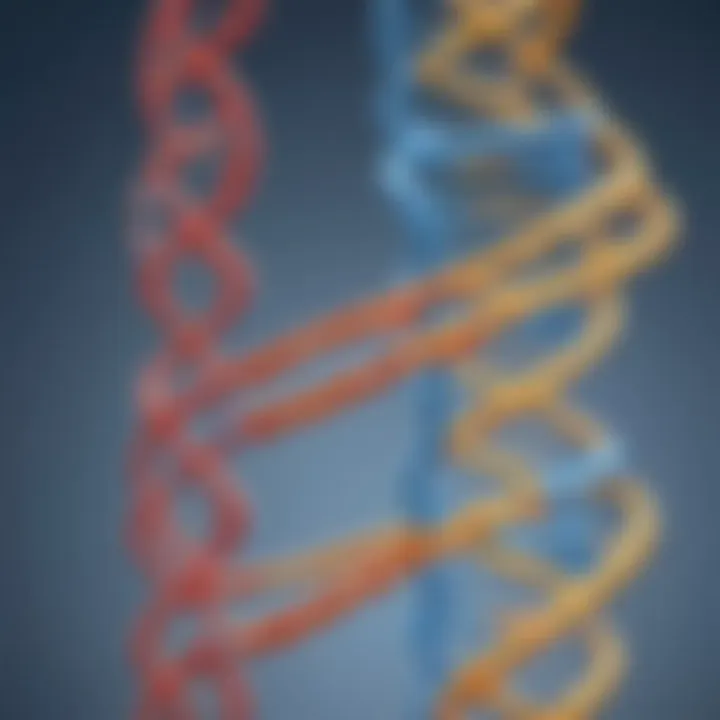
Thymine
Thymine, a pyrimidine base, boasts a simpler structure with a single-ring formation. Its primary role in DNA is to complement adenine. This base is unique to DNA, distinguishing it from RNA, which utilizes uracil in its place. The characteristic of thymine is that it can significantly affect the stability of the DNA molecule as it forms hydrogen bonds with adenine. Thymine's presence contributes to the overall fidelity of genetic information transmission, but changes or mutations in this base can lead to considerable genetic repercussions.
Cytosine
Cytosine is another essential pyramidine base. It's highly versatile, capable of forming multiple hydrogen bonds with its partner guanine. This pairing provides additional strength to the DNA structure, which is particularly important for segments of DNA subjected to environmental stress. Cytosine also plays a unique role in gene regulation processes, notably through methylation - a biochemical modification that can influence gene expression, further underscoring its significance beyond mere structure.
Guanine
As a purine, guanine is paramount in ensuring the structural balance of DNA. Pairing with cytosine, guanine also contributes to the strength of the genetic material. It features a unique ability to form three hydrogen bonds with cytosine, offering even more stability than adenine-thymine base pairs. This characteristic makes guanine a pivotal player in the configuration of genetic information, simultaneously impacting processes like gene expression and mutagenesis. Furthermore, guanine is involved in a variety of metabolic processes within the cell, showcasing its versatility and importance in molecular biology.
Understanding these bases provides a solid foundation for appreciating how DNA functions and its broader implications in genetics, cellular processes, and life as a whole.
The Pairing Rules of Nitrogenous Bases
In the intricate world of genetics, understanding the pairing rules of nitrogenous bases is key to grasping how DNA functions. At its core, these rules dictate how the bases interact, forming pairs that are crucial for the stability and integrity of the entire DNA structure. The beauty of these pairings lies in their specificity, which is fundamental to the processes of replication and transcription. By pairing in a predictable manner, the DNA ensures that the genetic information is preserved and accurately passed down from one generation to another.
The pairing rules specify that adenine pairs with thymine, while cytosine pairs with guanine. This complementary relationship not only strengthens the overall structure of DNA but also provides a mechanism for error correction during DNA replication. When bases link together, they allow the two strands of DNA to coil gracefully into a double helix, preserving the delicate balance necessary for genetic fidelity.
Complementary Base Pairing
Complementary base pairing is the cornerstone of DNA stability. Each pair forms hydrogen bonds that are both strong enough to hold the DNA strands together yet weak enough to allow the strands to separate during processes like replication. Here’s how it breaks down:
- Adenine (A) - always pairs with Thymine (T), forming two hydrogen bonds.
- Cytosine (C) - always pairs with Guanine (G), which creates three hydrogen bonds.
This clever design not only ensures the correct bases are paired but also contributes to the DNA's helical structure. If any bases were to mismatch, it could lead to mutations, which may or may not have significant effects on an organism's traits. In simpler terms, these pairing rules act like a lock and key, ensuring that only the right partners fit together.
"The pairing rules of nitrogenous bases ensure that the genetic information is not just kept safe but is also readily accessible when needed."
Hydrogen Bonds in Base Pairing
Diving deeper into the mechanics of base pairing, we must talk about the role of hydrogen bonds. These are weak intermolecular forces that, despite their fragility, play a massive role in maintaining the overall structure of DNA. Hydrogen bonds formed between adenine and thymine are somewhat weaker than those formed between cytosine and guanine. This difference in bond strength is significant when considering the processes DNA undergoes:
- Replicatoin: The ability of the strands to separate easily at the A-T rich sections of DNA is particularly useful during replication.
- Repair Mechanisms: Should some error or damage occur, the DNA repair machinery can access and fix the A-T regions more quickly than the G-C pairs.
Moreover, these hydrogen bonds allow the DNA to flexibly respond to changes in the cellular environment. In other words, while the backbone of DNA might be steadfast, the manner in which the bases interact gives life to the intricate dance of genetic information.
Overall, the pairing rules and the hydrogen bonds that support them are not mere technicalities; they're foundational elements that govern the functional narrative of DNA.
Chemical Composition of Bases
The chemical composition of the bases is a vital topic when we dive into the structure and function of DNA. The bases are the informational building blocks, the rungs of the DNA ladder, ensuring that genetic information is correctly copied, transmitted, and ultimately expressed in living organisms. Understanding their composition isn't just an academic endeavor; it highlights how life's intricacies arise from relatively simple chemical interactions and structures.
Structure of Nitrogenous Bases
The structure of nitrogenous bases can be intriguing. At their core, there are two main types: purines and pyrimidines. Each serves a unique purpose in the realm of DNA.
Purines vs Pyrimidines
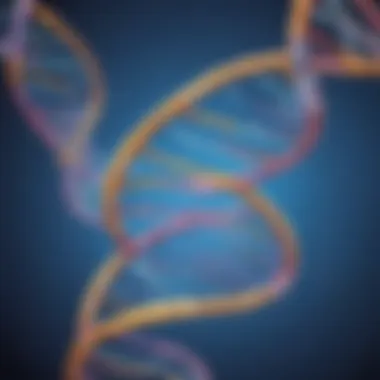
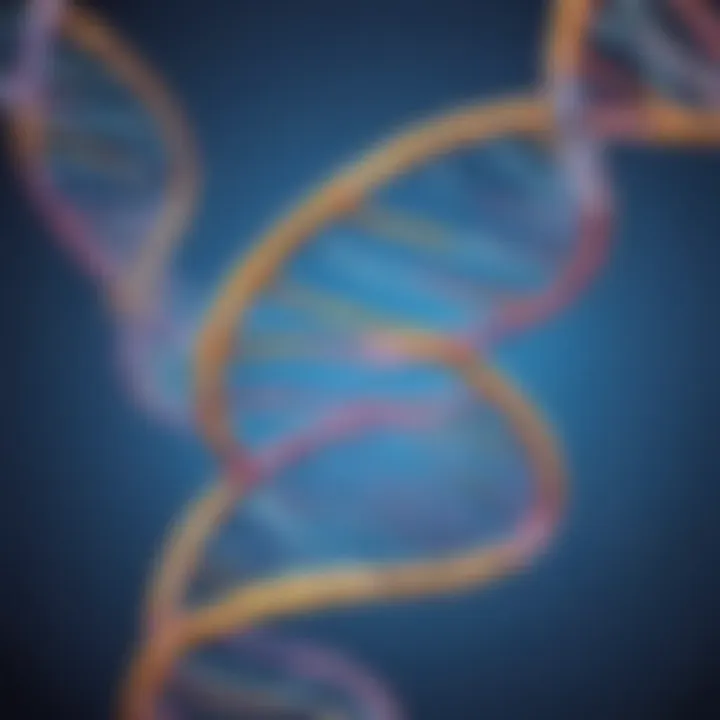
When comparing purines to pyrimidines, one observes that purines are larger molecules made up of a double-ring structure—an example being adenine and guanine. On the other side, pyrimidines, like cytosine and thymine, are smaller, characterized by a single-ring structure. This fundamental difference has implications.
- Key Characteristic: The bigness of purines allows them to interact in specific ways with the complementary pyrimidines during base pairing, which is crucial for DNA stability.
- Benefits: The structural diversity allows flexibility and resilience within the DNA molecule.
- Disadvantage: The complexity of purines might lead to mispairing if not accurately replicated, which can have downstream effects when genetic information is copied.
Functional Groups on Bases
Moving beyond structure, the functional groups attached to these bases are equally significant. They contribute to the chemical properties of the bases, influencing how they interact with one another in the DNA sequence. Each base has specific functional groups that take the lead in hydrogen bonding, describing how bases stick together.
- Key Characteristic: These groups can form hydrogen bonds with complementary bases, providing stability to the DNA structure.
- Benefits: The unique functional groups ensure precise base pairing, enhancing fidelity in genetic data transmission.
- Disadvantage: However, any alteration in these groups, such as through mutations or damage, can disrupt the entire sequence, possibly leading to genetic disorders or cancer.
Role of Sugar and Phosphate Backbone
Additionally, the sugar phosphate backbone plays a crucial role in DNA structure. The phosphates give the molecule a negative charge, while the sugar (deoxyribose) forms the central backbone. This structural relationship not only supports the nitrogenous bases but also makes the DNA molecule a stable, flexible chain capable of holding massive amounts of genetic data securely.
The balance between the bases and the backbone becomes a keystone in understanding how DNA functions in biological systems. Each part, no matter how small, plays a significant role in the overall integrity and history of life's code.
In essence, the chemical composition of bases and their configuration within the DNA structure underline the biological rhythms that sustain life.
Biological Significance of Base Pairing
Base pairing in DNA serves as the foundation for life as we know it. Without the correct pairing of nitrogenous bases, the very essence of genetic information would be compromised. The rigid structure of DNA can be likened to a well-designed blueprint; each base pair links together in a specific order to create the unique code for a living organism. These pairings help carry out key biological functions including cellular replication, gene expression, and protein synthesis.
"In the intricate world of genetics, base pairing is not just a detail; it's a cornerstone."
Genetic Information Encoding
At the heart of genetic inheritance lies the ability of DNA to encode information. Each sequence of bases—adenine, thymine, cytosine, and guanine—works like a language of its own. When these bases pair up, they form words and sentences that dictate characteristics passed down from parents to offspring. This is similar to how different combinations of letters can create countless words in a language.
- The Code: The sequence of the bases is translated into proteins through a process called transcription and translation. For instance, a particular sequence may instruct the cell to produce enzymes or build structural proteins like collagen.
- Variations Lead to Diversity: Slight changes in these base sequences can contribute to variations among individuals. This is how traits manifest, whether it be eye color or susceptibility to certain diseases.
- Influence of Base Pairing: The specificity of adenine pairing with thymine, and cytosine with guanine, is crucial in this encoding process. Any disruption in these pairings can lead to mutations, which sometimes result in genetic diseases or impacts on an organism’s functionality.
Impact on Protein Synthesis
Protein synthesis is another vital process strongly tied to base pairing. The blueprints laid out in DNA are converted into functional components, proteins, through a series of steps triggered by base interactions.
- Transcription: The DNA unwinds, and a complementary RNA strand is formed. If we think of DNA as a master recipe book, RNA is akin to a photocopy of a specific recipe that can be taken into the kitchen.
- Translation: The RNA is then translated into a specific protein with the help of ribosomes and transfer RNA (tRNA). This step is crucial because proteins perform a multitude of jobs in the cell, from speeding up reactions to providing structure.
- The Role of Base Pairing: Proper base pairing ensures that the correct sequences of amino acids are assembled during translation, thus producing functional proteins that are necessary for life.
Mutations and Their Effects on Base Pairing
Mutations are alterations in the DNA sequence and can have significant implications for how living organisms develop and function. In the context of our discussion on the structural composition of DNA, understanding mutations is crucial. They not only change how base pairs interact but can also lead to a variety of consequences, from benign variations to serious genetic disorders. This section aims to clarify both the types of mutations and their downstream effects on base pairing.
Types of Mutations
Point Mutations
Point mutations are specific changes to a single nucleotide base pair in the DNA sequence. This kind of mutation happens when a single chemical change occurs, such as an exchange of one base for another. A classic example is the switch of adenine for guanine. Point mutations stand out as they often do not alter the length of the DNA strand, making them a popular subject in the study of genetic variance.
The key characteristic of point mutations is that they can be classified further into three categories: silent, missense, and nonsense mutations.
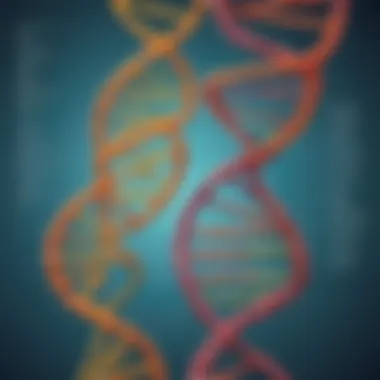
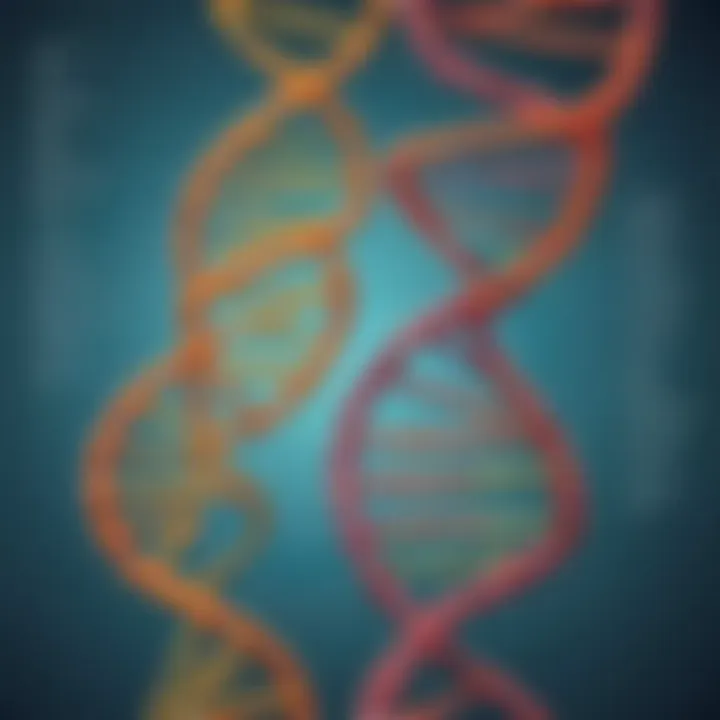
- Silent mutations change a base but do not alter the final amino acid produced, often because of the redundancy in the genetic code.
- Missense mutations change one amino acid in a protein, potentially altering its function. This could lead to beneficial, neutral, or harmful consequences.
- Nonsense mutations introduce a premature stop codon, which can truncate the resulting protein and potentially render it nonfunctional.
The unique feature of point mutations lies in their subtlety; even though they involve only a single base pair, they can have significant repercussions in genetic coding and protein synthesis. The advantage of studying point mutations is their relative simplicity, allowing researchers to grasp complex genetic ideas more easily.
Frameshift Mutations
Frameshift mutations, meanwhile, result from the insertion or deletion of one or more nucleotides. This shift in the reading frame of the DNA sequence changes how the whole sequence is interpreted. If you picture this as reading a sentence where a letter is added or removed, the meaning could be entirely lost after that point.
The primary characteristic of frameshift mutations is that they lengthen or shorten the DNA strand, leading to drastic alterations in the encoded protein that may affect its functionality.
A unique aspect of frameshift mutations is that they can cause significant changes in proteins that can result in serious diseases or disorders. Unlike point mutations, which may only affect one part of the protein, frameshifts can ripple through the genetic message, changing almost the whole product. While the consequence of such a mutation can often be detrimental, there are cases where frameshift mutations can lead to new functions that may either benefit or harm the organism.
Consequences of Mutations
Mutations can have a variety of outcomes depending on their nature and context.
- Neutral Effects: Often, mutations do not exert any noticeable impact, perhaps due to redundancy in the genetic code or their occurrence in non-coding regions of DNA.
- Beneficial Effects: In some cases, mutations can equip organisms with advantageous traits that can provide a survival edge over others, contributing to the gradual process of evolution.
- Harmful Effects: Unfortunately, some mutations lead to genetic disorders or diseases, disrupting normal biological functions. For example, cystic fibrosis or sickle cell disease emerge due to significant mutations in genes affecting essential proteins.
In summary, mutations serve as critical players in the ever-evolving tapestry of life. While they can foster diversity and adaptability, the reality of their consequences cannot be dismissed, especially when it comes to human health. Understanding the intricacies of mutations is essential for anyone interested in genetics, medicine, and the broader implications for biodiversity and conservation.
"Mutations can be like a double-edged sword, offering both opportunities for change and risks of dysfunction."
Recent Advances in DNA Research
The field of DNA research has seen remarkable strides in recent years, marking a significant chapter in our understanding of genetics and molecular biology. Advances in technology have streamlined various methods for studying and manipulating DNA, which, in turn, holds promise for innovative solutions in medicine, agriculture, and environmental sustainability. \n\n> "Understanding DNA paves the path for groundbreaking therapeutic approaches and enhances our ability to fight genetic diseases."
One of the key elements of these advances is the increasing success of gene editing technologies. By employing these tools, scientists can directly modify the genetic fabric of organisms. This is not just a lofty ambition but a practical application with profound implications for our daily lives. \n\n### Gene Editing Technologies
Gene editing technologies, notably CRISPR-Cas9, have revolutionized how we approach genetic modification. This technique acts like molecular scissors, allowing researchers to cut DNA at specific locations and make precise changes. It's akin to editing a document; if a sentence doesn’t convey the intended meaning, one can edit or substitute it for clarity.
\nThe ease and efficiency of CRISPR systems are unparalleled. Through its applications, we can:
- Target specific genes for alteration, which can lead to disease correction.
- Insert or delete genetic material, enhancing crop yields or resistance to pests in agriculture.
- Correct mutations that lead to genetic disorders in humans, opening doors to potential cures.
\nWhile the technology holds enormous potential, ethical considerations are deeply woven into discussions about its use. As we venture into editing the very essence of life, we must also ponder the ramifications of such power. The capacity to alter human DNA, for instance, brings forward important dialogues regarding gene therapy, heritability, and consent.
\n### Implications for Medicine and Biotechnology
The implications of these recent advancements are profound. In medicine, the ability to edit genes provides a pathway to treat or prevent numerous diseases, ranging from simple inherited conditions to complex illnesses like cancer. The prospect of personalized medicine is becoming more attainable, allowing treatment plans to be tailored to an individual's genetic makeup. \n\nAdditionally, biotechnology stands to benefit from these developments through enhanced agricultural practices and food security. Genetic modifications can lead to crops that require less water and can withstand harsh environments. This can contribute significantly to food production in regions battling adverse climate conditions. \n\nIn summary, advancements in DNA research and gene editing technologies offer transformative benefits across multiple sectors. Not only do they enhance our understanding of genetics, but they also enable us to address pressing global issues including health disparities and food scarcity. As we sail into this new era of DNA exploration, it’s essential we navigate these waters thoughtfully, weighing benefits against ethical considerations to ensure a responsible and equitable approach to genetic innovation.
Culmination and Future Directions
The exploration of DNA's structural composition, particularly the ladder rungs that form its base, brings understanding to the very essence of life. This article highlighted the intricacies of nitrogenous bases and their critical roles in genetic information storage and transfer. Without these bases pairing precisely, the genetic code could not be reliably copied or expressed, making their study essential to both science and medicine. The understanding of these components extends beyond textbooks, impacting real-world applications such as gene editing and genetic therapies.
Key points surrounding DNA's rungs include:
- The complementary base pairing (A with T and C with G) which ensures the fidelity of genetic information.
- Insights into mutations, which can arise naturally or due to environmental factors, challenge our understanding of heredity and disease.
- Advances in technology allow scientists to modify these sequences, with profound implications everywhere from agriculture to human health.
"Understanding how DNA works is not just about biology; it's about grasping the blueprint of life itself, providing insight into everything from evolution to potential cures for cancer."
In terms of future directions, research is moving swiftly towards refining methodologies used in gene editing, such as CRISPR. This technology holds the promise of correcting genetic disorders and improving crop resistance, but it comes with ethical considerations. As we continue to unlock the secrets contained within DNA's structure, we must tread carefully respecting both the scientific possibilities and the ethical ramifications these advancements carry.
Summarizing Key Findings
When diving deep into the structure of DNA, it's clear that the rungs built from nitrogenous bases are more than just simple structures. They are intricate pairs essential to life's processes. Understanding their function can illuminate not just how life perpetuates, but also how it can be modified.
- Complementary Base Pairing: This is a fundamental rule that ensures accurate replication of DNA, meaning that offspring inherit stable and predictable traits.
- Role of Mutations: Mutations can bring about changes that fuel evolution or cause diseases, making them a double-edged sword in genetic studies.
- Advances in Biotechnology: The fast-paced progress in technology directly correlates with how we can influence these base pairs for better health and agriculture.
The Future of DNA Research
Looking ahead, the trajectory of DNA research holds exciting promise. Scientists are not only refining current technologies but also seeking to understand the epigenetic factors that can influence gene expression without altering the underlying DNA sequence.
- Gene therapies have a bright future as potential treatments for genetic disorders, with ongoing clinical trials paving the way for breakthrough therapies.
- Research into synthetic biology opens possibilities for creating new life forms or engineered organisms with specific traits.
- There's a growing need for comprehensive bioinformatics tools to manage and analyze the vast amounts of data generated, enabling discoveries and potential applications in real-time.
Ongoing dialogue about ethics in genetic technologies will also shape the future landscape. As we stand at this crossroads, the potential for knowledge and innovation seems boundless, poised to revolutionize how we understand life as we know it.
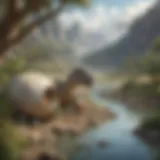
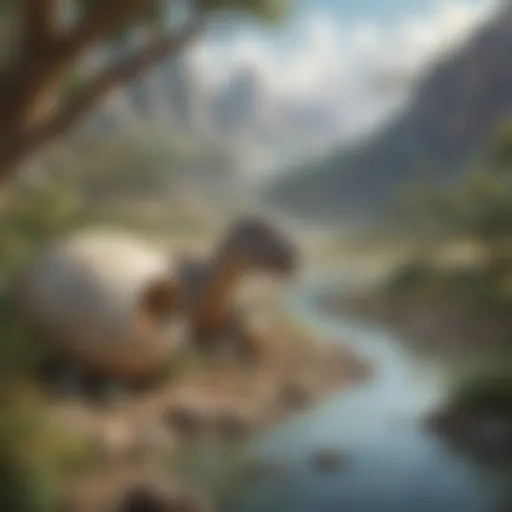